Ductility, strain, and toughness represent foundational principles within the domain of engineering, particularly within the realms of materials science and mechanical engineering.
Ductility signifies the propensity of a material to undergo plastic deformation prior to fracture. It quantifies the material’s capacity to sustain significant elongation or bending without rupture. Materials exhibiting high ductility, such as copper and aluminum, can undergo extensive deformation before reaching failure, in contrast to brittle materials like glass or ceramics, which exhibit minimal deformation prior to fracture.
Strain denotes the extent of deformation experienced by a material per unit length in response to applied stress. It characterizes the degree of elongation or compression experienced by the material. Typically expressed as a ratio of the change in length to the original length, strain elucidates the extent of mechanical deformation incurred by the material under external forces.
Toughness serves as a metric for a material’s resilience against fracture when subjected to stress. It quantifies the energy required to initiate fracture within the material and is commonly measured in joules per cubic meter (J/m^3). Materials possessing high toughness can absorb substantial amounts of energy prior to fracturing, rendering them well-suited for applications necessitating robust impact resistance, such as structural components in construction or protective gear.
In essence, ductility delineates a material’s deformability prior to failure, strain elucidates the extent of mechanical deformation per unit length, and toughness quantifies a material’s ability to withstand fracture under applied stress.
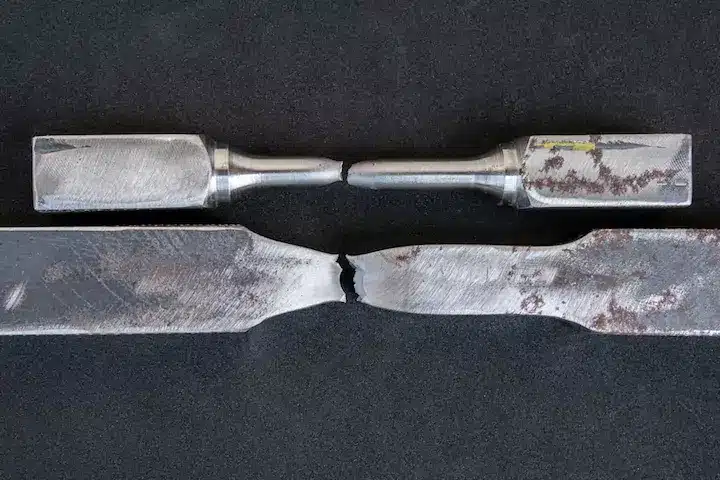
Examining Toughness and Hardness within Material Science
Toughness and hardness are fundamental properties within the domain of material science, offering distinct insights into a material’s behavior under various conditions.
Hardness refers to a material’s resistance to indentation or scratching, typically quantified through standardized tests such as the Mohs scale or the Brinell, Vickers, or Rockwell hardness tests. It reflects the material’s ability to withstand localized deformation when subjected to external forces. Materials with high hardness exhibit greater resistance to deformation, making them suitable for applications requiring durability and wear resistance.
Toughness, on the other hand, embodies a material’s ability to absorb energy and deform plastically before fracturing. It is a comprehensive measure that considers both strength and ductility, encompassing the energy required to initiate and propagate cracks within the material. Toughness is often evaluated through tests such as Charpy or Izod impact tests, which assess a material’s resistance to sudden and dynamic loading. Materials with high toughness can absorb significant amounts of energy before failure, making them suitable for applications where resilience to impact and fracture is paramount.
While hardness and toughness are distinct properties, they are not mutually exclusive. Some materials may exhibit high hardness and toughness simultaneously, while others may prioritize one property over the other depending on the intended application. Understanding the interplay between hardness and toughness is crucial in selecting materials for specific engineering purposes, ensuring optimal performance and reliability in diverse operating environments.
Relationship Between Ductility and Toughness in Materials
The interplay between ductility and toughness in materials is multifaceted, contingent upon various factors including material composition, loading conditions, and temperature. Broadly, materials exhibiting higher ductility typically demonstrate greater toughness, as they possess the capacity to absorb more energy prior to fracture.
Nevertheless, it is conceivable for a material to exhibit high ductility yet low toughness, or conversely, high toughness but low ductility. For instance, certain metals like aluminum exemplify both high ductility and toughness, rendering them suitable for applications necessitating these dual attributes. Conversely, materials such as cast iron may exhibit high ductility but fracture with minimal deformation, showcasing brittleness despite their ductile nature.
Furthermore, the correlation between ductility and toughness can be modulated by temperature fluctuations. Some materials may transition to a more brittle state at lower temperatures, while others may become more ductile. For instance, ceramics may demonstrate increased brittleness at low temperatures, whereas elastomers might exhibit enhanced ductility under the same conditions.
Engineers and materials scientists routinely evaluate both ductility and toughness when selecting materials for specific applications, recognizing their pivotal roles in determining a material’s resistance to stress and deformation. Achieving an optimal balance between ductility and toughness is contingent upon the precise requirements of the intended application.
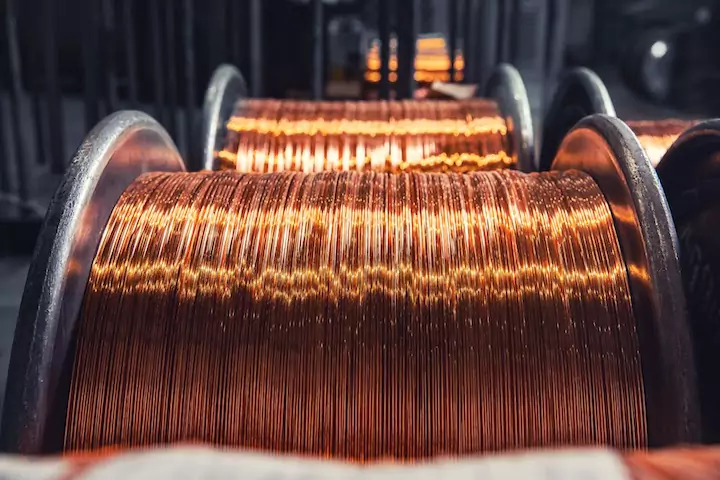
Stress strain curve of ductile materials
The stress-strain curve of ductile materials presents a distinctive profile characterized by several key features. Initially, as stress is applied, ductile materials exhibit a linear relationship between stress and strain, indicative of elastic deformation. This linear region, known as the elastic region, delineates the material’s ability to return to its original shape once the stress is removed, underscoring its resilience within this domain.
Subsequently, as the stress intensifies, ductile materials enter a phase termed the plastic region, wherein they manifest significant deformation without fracture. This phase is marked by a gradual increase in strain with little to no increase in stress, exemplifying the material’s plasticity and capacity for extensive deformation.
The stress-strain curve of ductile materials culminates at the point of ultimate tensile strength (UTS), denoting the maximum stress the material can withstand before fracture. Beyond this point, the material undergoes necking, a localized reduction in cross-sectional area, leading to eventual rupture.
Importantly, the area under the stress-strain curve up to the UTS encompasses the material’s resilience, reflecting its capacity to absorb energy prior to yielding. Ductile materials, characterized by their ability to undergo significant plastic deformation before failure, typically exhibit higher resilience compared to brittle counterparts.
Ductility vs. Brittleness in Materials Science
In materials science, the concepts of ductility and brittleness serve as fundamental descriptors delineating the behavior of materials under stress. Ductility refers to the propensity of a material to undergo plastic deformation before fracture, whereas brittleness denotes a material’s tendency to fracture without significant plastic deformation.
Ductile materials, such as many metals and some polymers, exhibit notable elongation and deformation under stress, often characterized by the ability to be drawn into wires or hammered into thin sheets without fracturing. This behavior arises from the ability of the material’s atomic structure to undergo rearrangement and sliding past each other, thereby accommodating the applied stress.
In stark contrast, brittle materials, including ceramics and certain alloys, tend to fracture abruptly with minimal plastic deformation when subjected to stress beyond their yield point. The fracture in brittle materials typically occurs along cleavage planes or grain boundaries, resulting in the rapid propagation of cracks throughout the material.
The distinction between ductility and brittleness holds significant implications for various engineering applications. Ductile materials are preferred for structures or components subjected to dynamic or cyclic loading, as they can withstand deformation without catastrophic failure. Conversely, brittle materials are often utilized in applications where stiffness and resistance to deformation are prioritized over ductility, such as in certain electronic components or protective coatings.
Difference Between Ductility and Toughness
Ductility and toughness are distinct mechanical properties crucial for understanding material behavior, especially under stress conditions.
Ductility pertains to a material’s capacity for plastic deformation prior to fracture, signifying its ability to undergo significant elongation or bending without rupturing. Materials exhibiting high ductility can sustain considerable deformation before reaching failure, whereas brittle materials tend to fracture abruptly with minimal deformation.
Conversely, toughness refers to a material’s resistance to fracture when subjected to external forces. It quantifies the energy absorption capability of a material before failure and is typically expressed in joules per cubic meter (J/m^3). Tough materials demonstrate resilience against fracture and are preferred in applications demanding impact resistance, such as structural components and safety gear.
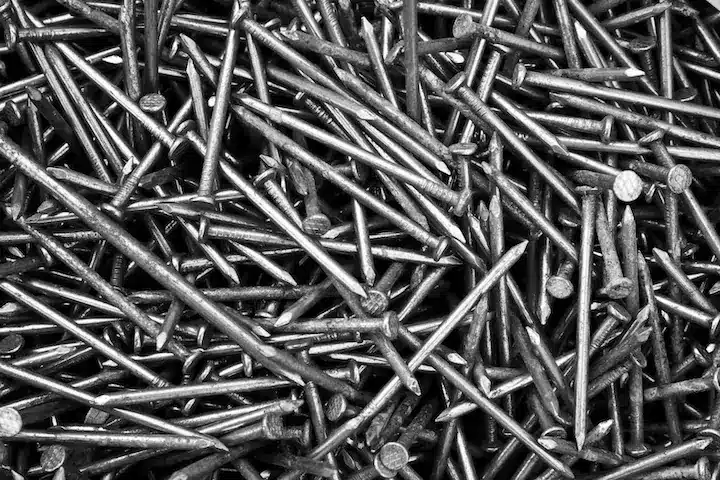
Materials Mechanics Theory
Materials mechanics theory is a pivotal domain within materials science, dedicated to comprehending the response of materials to external forces. This encompasses an intricate analysis of material deformation, encompassing both elastic and plastic behaviors, stress-strain dynamics, and fracture mechanics.
The mechanical performance of materials is intricately intertwined with atomic and molecular interactions within the material structure. Factors such as atomic bonding, crystalline arrangement, defects, impurities, and environmental conditions collectively influence mechanical properties.
Engineers and materials scientists employ a spectrum of theoretical frameworks to elucidate mechanical phenomena:
- Linear Elasticity Theory: This framework postulates elastic material behavior, wherein deformation is directly proportional to applied stress, reverting to the original state upon stress removal.
- Plasticity Theory: Concerned with permanent deformation beyond elastic limits due to high stress levels.
- Fracture Mechanics: Focuses on predicting material failure under diverse loading conditions, facilitating comprehension of fracture mechanisms.
- Fatigue Theory: Explores material response to cyclic loading, crucial for mitigating fatigue-induced failures.
- Creep Theory: Addresses gradual material deformation under sustained stress, particularly relevant in high-temperature and long-term loading scenarios.
In essence, materials mechanics theory is an interdisciplinary pursuit aimed at unraveling material behaviors under external forces, thereby informing material selection and design for optimal performance and durability across various applications.
Formula for Toughness
Toughness, a fundamental material property, delineates a material’s capacity to absorb energy prior to fracturing, denoted typically in joules per cubic meter (J/m^3). The determination of toughness hinges upon the mode of loading to which the material is subjected.
Under tensile loading conditions, the formula for toughness derives from the integral of the stress-strain curve. Therein, toughness manifests as the area under the stress-strain curve up to the fracture point:
T =∫(σdϵ)
Here, T signifies toughness, σ represents stress, and ε signifies strain.
For scenarios of impact loading, the formula for toughness corresponds to the energy requisite for material fracture. Here, toughness emerges from the total energy dissipated by the material during the impact test:
T=∫(Pdt)
Where T denotes toughness, P signifies the applied force during the impact, and t denotes time.
Multiple factors, including temperature, loading rate, presence of defects and impurities, and the nature of loading (tensile or impact), can influence toughness. The optimization of toughness alongside other material attributes, such as ductility and strength, necessitates consideration of the specific demands of the application.
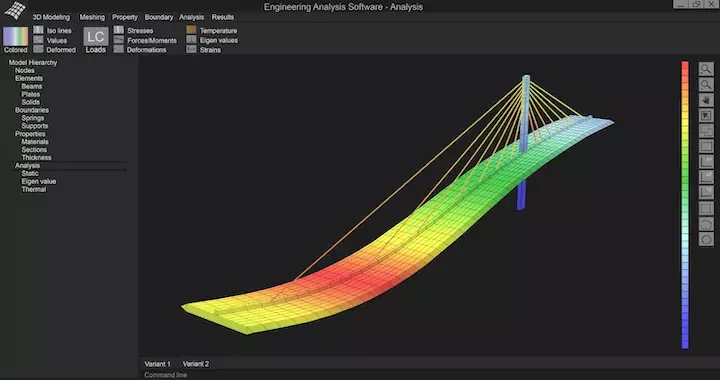
Distinguishing High Toughness from High Ductility
High toughness and high ductility are distinct material characteristics with nuanced implications in various applications, and their interrelation is not straightforward. While both attributes are desirable, they represent different aspects of material behavior.
High toughness signifies the capacity of a material to absorb substantial energy prior to fracture, rendering it suitable for scenarios demanding resistance to impact or sudden loads, such as structural components or protective gear.
Conversely, high ductility denotes the ability of a material to undergo plastic deformation before rupture, enabling it to bend or stretch without catastrophic failure, as observed in applications like wire or thin sheet fabrication.
It’s noteworthy that certain materials exhibit both high toughness and high ductility concurrently. Notably, certain steel alloys possess a blend of superior strength, ductility, and toughness, offering versatility across a spectrum of applications.
In essence, while high toughness and high ductility are distinct attributes, they collectively influence a material’s overall performance in specific applications. Achieving an optimal equilibrium between these properties necessitates a tailored approach, accounting for the precise requirements of each application.
Distinguishing Between Strain and Ductility
In the realm of materials science and engineering, strain and ductility represent distinct facets of a material’s response to stress. Strain quantifies the degree of deformation induced by an external force, defined as the change in length relative to the original length:
ε = ΔL / L0
where ε denotes strain, ΔL signifies the change in length, and L0 represents the initial length.
Ductility characterizes a material’s capacity for plastic deformation prior to fracture. It reflects the material’s pliability or its ability to undergo stretching or bending without rupturing. Ductility is typically articulated as a percentage elongation, computed as the increase in length post-fracture relative to the original length:
% elongation = (Lf – L0) / L0 * 100%
where Lf denotes the length of the material after fracture.
Strain denotes the deformation resulting from applied stress, while ductility gauges a material’s plastic deformation capability prior to failure. These concepts intertwine as a material’s ductility is intricately linked to the extent of strain it can endure before rupture.
Factors Affecting Material Toughness
Numerous variables impact the toughness of a material, including:
- Chemical Composition: The elemental makeup of a material significantly influences its toughness. For instance, the incorporation of specific elements, like carbon in steel, can enhance strength while potentially compromising toughness.
- Microstructural Characteristics: The size, morphology, and dispersion of microstructural constituents, such as grain boundaries, second phases, and inclusions, play a crucial role in determining material toughness. A refined microstructure often correlates with improved toughness due to more uniform stress distribution.
- Temperature: Material toughness is sensitive to temperature variations. Elevated temperatures can lead to reduced toughness owing to diminished strength and heightened ductility.
- Loading Rate: The rate at which a material experiences mechanical loading impacts its toughness. Higher loading rates typically result in decreased toughness due to reduced time for deformation processes.
- Environmental Conditions: Environmental factors, such as exposure to corrosive agents, elevated temperatures, and radiation, can degrade material toughness over time.
- Processing History: The manufacturing processes undergone by a material, such as casting, forging, or heat treatment, influence its toughness. Certain heat treatments, for example, can refine the microstructure, enhancing toughness.
In summary, material toughness is a complex interplay of chemical composition, microstructure, temperature, loading rate, environmental exposure, and processing methods. Achieving an optimal balance between toughness and other material properties necessitates a thorough understanding of application-specific requirements.
Does the Increase in Hardness Correlate with Enhanced Toughness?
Hardness and toughness are distinct material properties, each influenced by various factors. It is crucial to recognize that augmenting hardness does not invariably translate to an enhancement in toughness.
Hardness denotes a material’s resistance to indentation, often utilized as a gauge of its strength. This property can be bolstered by fortifying the material, such as through alloying steel with additional elements.
Conversely, toughness gauges a material’s capacity to absorb energy before fracturing, a composite measure of strength and ductility. It primarily signifies a material’s resilience against impact.
In certain scenarios, heightening a material’s hardness may inadvertently diminish its toughness. For instance, fortifying a steel alloy to amplify its strength and consequently its hardness might also compromise its ductility and overall toughness.
Optimal hardness-toughness equilibrium hinges on the specific application requirements. For instances necessitating robust impact resistance, prioritizing materials with heightened toughness, even at the expense of reduced hardness, may be warranted. Conversely, in applications mandating elevated hardness, materials with superior hardness, albeit lower toughness, may be more appropriate.
How Does Strain Correlate with Ductility in Materials Science?
In materials science and engineering, the relationship between strain and ductility is fundamental to understanding a material’s response to external forces. Strain denotes the degree of deformation induced by applied stress, quantified as the change in length relative to the original length of the material:
ε = ΔL / L0
Where ε represents strain, ΔL is the change in length, and L0 is the initial length.
Ductility, conversely, characterizes a material’s capacity for plastic deformation prior to fracture, reflecting its ability to undergo shaping processes like stretching or bending without fracturing. Typically expressed as a percentage elongation, ductility is calculated as the increase in length post-fracture divided by the original length:
% elongation = (Lf – L0) / L0 * 100%
Here, Lf denotes the length after fracture.
The interconnection between strain and ductility arises from their shared emphasis on material deformation under stress. Enhanced ductility implies a material can sustain greater plastic deformation before failure, indicating a higher tolerance for strain. Conversely, materials with limited ductility exhibit reduced deformation capacity before failure, indicating a lower threshold for strain.
Strain measures the extent of material deformation under stress, while ductility gauges its ability to undergo plastic deformation before fracturing. These concepts are interconnected, as a material’s ductility directly influences the magnitude of strain it can endure before failure.
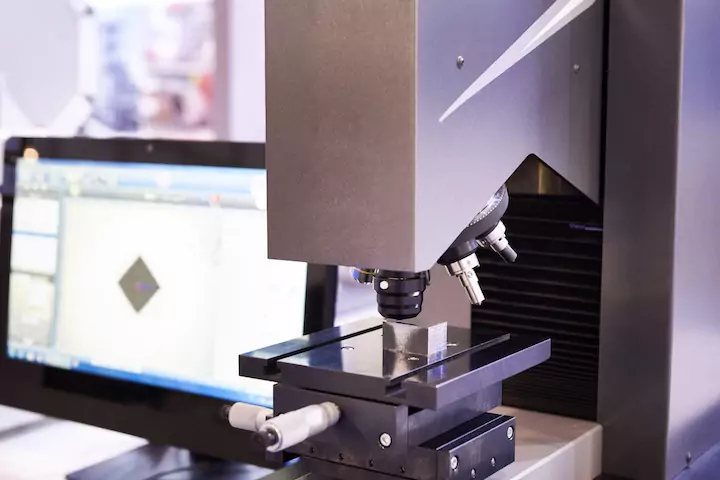
Analyzing Resilience Utilizing the Stress-Strain Relationship
Resilience, within the realm of materials science, delineates a crucial property denoting the capability of a substance to absorb energy before succumbing to deformation. Specifically contextualized within the stress-strain curve, resilience is determinable as the integral area beneath the curve up to the point of yielding.
The pivotal juncture on the stress-strain curve, termed the yield point, signifies the onset of material yielding or permanent distortion. Quantitatively, the resilience of the material correlates with the energy requisite for deforming it to the yield point.
The determination of a material’s resilience from its stress-strain profile entails the utilization of the formula:
R = (1/2) * σy * εy
Here, R signifies resilience, while σy and εy represent the yield stress and yield strain, respectively.
The parameters of yield stress and yield strain are discernible from the stress-strain curve, facilitating the calculation of resilience as the product of yield stress and yield strain divided by two.
In essence, materials characterized by high resilience exhibit augmented capacity to absorb energy before yielding, indicative of their ability to withstand elevated stress levels before incurring permanent deformation. Conversely, materials endowed with low resilience manifest diminished capability to absorb energy, rendering them susceptible to permanent deformation under comparatively lower stress levels.
Produce High-Quality Components Efficiently – Initiate Your Next Project with BOYI
For precision manufacturing solutions encompassing CNC machining, injection molding, 3D printing, or urethane casting, BOYI stands as your premier resource for custom and intricate mechanical components. Leveraging our expertise in engineering and materials science, we assist in material selection tailored to your specific requirements and seamlessly navigate you through the manufacturing workflow to ensure optimal outcomes.
Embark on your project today by registering an account and submitting your design to experience the full capabilities of our instant online CNC quotation system, Design for Manufacturability (DFM) feedback, and intelligent platform. With BOYI, expect expedited delivery of complex components without compromising on quality.